Scientists finally discover why photosynthesis is so efficient
Life on earth owes its existence to the light-absorbing ability of photosynthetic organisms, from microscopic bacteria to majestic sequoias
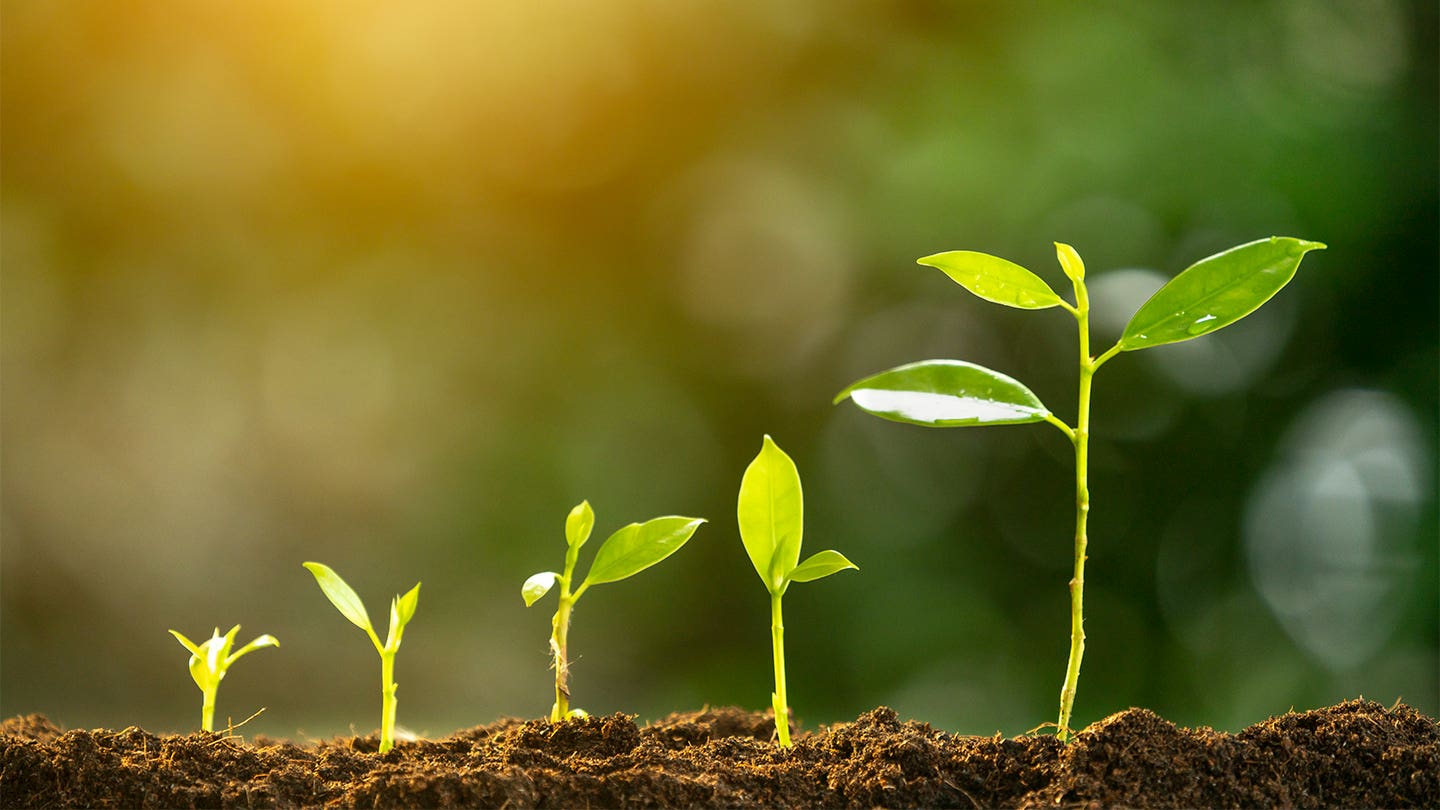
[July 4, 2023: JJ Shavit, The Brighter Side of News]
Photosynthesis is a fundamental process that sustains life on Earth, enabling plants to convert light into energy. (CREDIT: Creative Commons)
Life on earth owes its existence to the incredible light-absorbing ability of photosynthetic organisms, from microscopic cyanobacteria to majestic sequoias. The mechanism that powers this biological engine—photosynthesis—transforms sunlight into chemical energy with astounding proficiency.
Central to this process is a network of light-harvesting proteins within cells that soak up energy from the sun. But how does this finely tuned mechanism achieve such high efficiency? New research from a team of chemists at the Massachusetts Institute of Technology (MIT) offers a fresh perspective, revealing that a chaotic, disordered arrangement of these proteins may be the secret behind their remarkable performance.
The complex dance of photosynthesis begins when cells absorb light from the sun, each burst of energy—known as a photon—navigating an intricate relay race among a sequence of light-harvesting proteins. The end goal of this energetic expedition is the photosynthetic reaction center.
This biological hot spot, where energy becomes electrons, subsequently powers the creation of sugar molecules. These sugars, the primary fuel for life, are the end product of an incredible chain of events that starts with the sun’s rays and ends with the nourishment of life as we know it.
Related Stories
Under the microscope, this energy transfer unfolds with near-perfect efficiency: almost every photon absorbed by a photosynthetic cell generates an electron, an achievement known as near-unity quantum efficiency. But until now, the specifics of how proteins within the light-harvesting complex—the antenna—reach such lofty heights of performance have remained shrouded in mystery.
Now, groundbreaking work by an MIT research team led by Associate Professor of Chemistry Gabriela Schlau-Cohen provides exciting insights into this phenomenon. Schlau-Cohen explains, “In order for that antenna to work, you need long-distance energy transduction. Our key finding is that the disordered organization of the light-harvesting proteins enhances the efficiency of that long-distance energy transduction.” This discovery, showcasing the hidden advantages of disorder, is the fruit of the team's labor to measure energy transfer between light-harvesting proteins for the first time.
The research, set to appear in the prestigious Proceedings of the National Academy of Sciences, is a collaborative effort involving lead authors and MIT postdocs Dihao Wang and Dvir Harris, former MIT graduate student Olivia Fiebig, and Professor of Chemistry Jianshu Cao. Together, they offer a fresh take on energy capture, the first step in photosynthesis.
For the first time, MIT chemists have measured the energy transfer between photosynthetic light-harvesting proteins, allowing them to discover that the disorganized arrangement of light-harvesting proteins boosts the efficiency of the energy transduction. (CREDIT: Courtesy of the researchers)
The team centered their study on purple bacteria, frequently encountered in oxygen-deficient aquatic habitats. These microbes offer an accessible model for photosynthetic research because of their unique light-harvesting mechanisms. At the heart of each purple bacterium are light-harvesting complexes composed of proteins and light-absorbing pigments like chlorophyll, where photons embark on their journey towards the reaction center.
Previously, scientists have managed to probe how energy maneuvers within a single protein using ultrafast spectroscopy—a technique that deploys incredibly brief laser pulses to capture events that occur within femtoseconds to nanoseconds. However, tracking the energy's route between proteins proved considerably more challenging, requiring a strategy to position multiple proteins in a controlled manner.
By varying the diameter of the nanodiscs, researchers replicated a range of distances between the proteins. The closest distance possible between neighboring LH2, which is the most common in native membranes, is 25 Å and resulted in a timescale of 5.7 ps. Larger distances of 28 to 31 Å resulted in timescales of 10 to 14 ps. (CREDIT: PNAS)
In a remarkable feat of bioengineering, the MIT scientists fashioned synthetic nanoscale membranes—nanodiscs—that mimic the composition of naturally occurring cell membranes. By manipulating the size of these nanodiscs, they gained control over the spacing between two proteins embedded within the discs. Schlau-Cohen's team integrated two forms of the primary light-harvesting protein found in purple bacteria—known as LH2 and LH3—into their synthetic nanodiscs. LH2 operates under normal light conditions, while LH3, a variant, comes into play in low light scenarios.
Using the advanced cryo-electron microscope at the MIT.nano facility, the team imaged their membrane-embedded proteins, demonstrating that they were placed at distances similar to those in natural membranes. This setup allowed them to gauge the distances between the light-harvesting proteins, which measured between 2.5 to 3 nanometers.
Having positioned the proteins, the researchers turned their attention to the energy transfer between them. As LH2 and LH3 absorb slightly different wavelengths of light, the team could track the energy transfer between these proteins using ultrafast spectroscopy. They found that when proteins were closer together, it took a mere 6 picoseconds for a photon to complete its journey from one protein to the other. If the proteins were farther apart, this trip extended up to 15 picoseconds.
This observation underscored that the faster the photon completes its trip, the more efficient the energy transfer becomes, reducing the risk of energy loss during transit. Schlau-Cohen elucidates this point, noting, “When a photon gets absorbed, you only have so long before that energy gets lost through unwanted processes such as nonradiative decay, so the faster it can get converted, the more efficient it will be.”
Unexpectedly, the team discovered that proteins arranged in a regular lattice structure didn't perform as well as their counterparts in randomly arranged structures, a configuration typically observed in living cells. This intriguing finding led Schlau-Cohen to ponder: “Ordered organization is actually less efficient than the disordered organization of biology, which we think is really interesting because biology tends to be disordered. This finding tells us that that may not just be an inevitable downside of biology, but organisms may have evolved to take advantage of it.”
With their pioneering methodology now allowing for the measurement of inter-protein energy transfer, the MIT team plans to examine energy exchange between other proteins. This includes studying the transfer from antenna proteins to reaction center proteins and investigating energy transfer in photosynthetic organisms beyond purple bacteria, such as green plants.
Their groundbreaking work has shed new light on the fundamental mechanisms of photosynthesis and opened avenues for deeper explorations into this fascinating world of light, life, and the beautifully orchestrated chaos that connects them.
Note: Materials provided above by the The Brighter Side of News. Content may be edited for style and length.
Like these kind of feel good stories? Get the Brighter Side of News' newsletter.
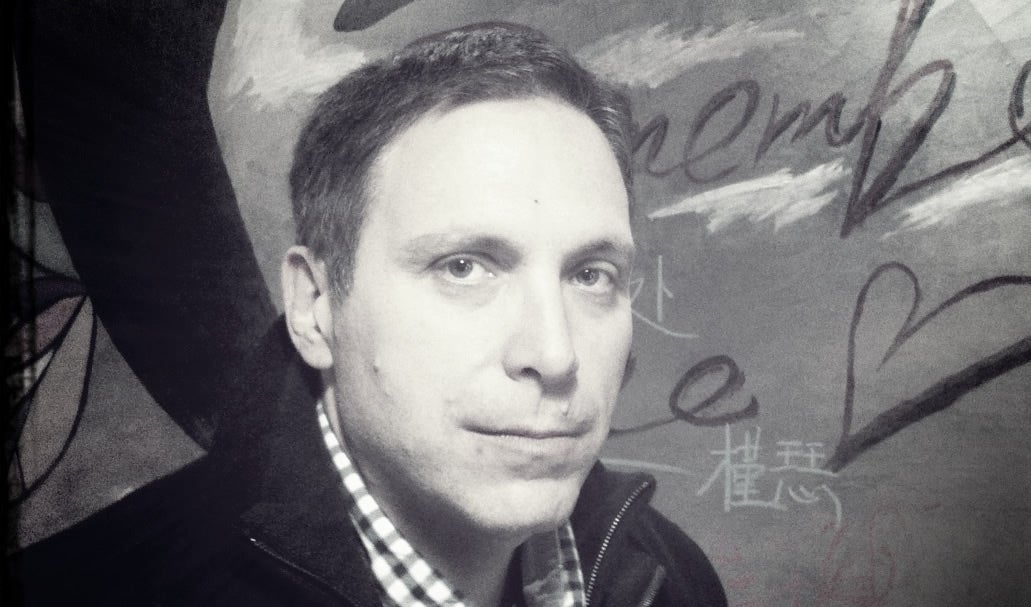
Joseph Shavit
Head Science News Writer | Communicating Innovation & Discovery
Based in Los Angeles, Joseph Shavit is an accomplished science journalist, head science news writer and co-founder at The Brighter Side of News, where he translates cutting-edge discoveries into compelling stories for a broad audience. With a strong background spanning science, business, product management, media leadership, and entrepreneurship, Joseph brings a unique perspective to science communication. His expertise allows him to uncover the intersection of technological advancements and market potential, shedding light on how groundbreaking research evolves into transformative products and industries.