Silicon qubits bring scalable quantum computing closer to reality
Silicon spin qubits could enable faster, scalable quantum computing with high fidelity and longer coherence at higher temperatures.
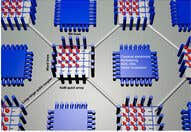
Silicon spin qubits are showing promise for building fast, scalable quantum computers that can operate at higher temperatures with high accuracy. (CREDIT: Intelligent Computing)
Silicon spin qubits could soon help unlock the next stage of quantum computing. For decades, scientists have tried to harness the power of quantum mechanics to perform calculations beyond the reach of classical computers. That dream may now be closer than ever.
Quantum computers work in a completely different way from the devices you use every day. Instead of processing information with bits that are either 0 or 1, quantum computers use quantum bits—or qubits—that can be both at once, thanks to a strange property called superposition. They also take advantage of quantum entanglement and interference, which allow qubits to interact and influence one another in ways no classical system can match.
These tools make it possible for quantum computers to perform some tasks much faster than today’s supercomputers. Researchers hope quantum technologies will help solve complex problems in drug discovery, economics, encryption, and even climate science. Many in the field believe that quantum computing could spark a revolution across science and industry.
From Concept to Prototype
After 40 years of work, quantum systems are now entering what scientists call the NISQ era—short for noisy intermediate-scale quantum. In this stage, small quantum processors can already perform certain calculations better than classical ones, even if they’re still prone to error. Experiments with superconducting qubits and photonics have shown clear quantum advantages. Some platforms even operate near the threshold needed for fault-tolerant computing.
But building a universal quantum computer—one that can handle any task—is much harder. That goal could require millions of physical qubits. Today’s systems offer only a few hundred. Closing this gap remains a major challenge, especially when it comes to coupling qubits across long distances and storing quantum states for long periods. Bulky electronics and complex cooling systems also add to the problem.
One approach gaining momentum comes from a familiar material: silicon. The same material used in traditional computer chips might also lead the way for quantum ones.
Related Stories
Why Silicon Spin Qubits Stand Out
A recent review published in Intelligent Computing, a Science Partner Journal, outlines how silicon spin qubits are shaping up as one of the most promising paths toward large-scale quantum computers. The review, titled “Single-Electron Spin Qubits in Silicon for Quantum,” summarizes the rapid progress in this area and the challenges that still remain.
Silicon spin qubits store quantum information using the spin of an electron—a quantum version of a tiny magnet. These spins are confined in tiny structures called quantum dots, sometimes described as “artificial atoms.” Researchers can trap and control single electrons inside these dots using electric fields or magnetic signals.
What makes silicon spin qubits especially attractive is their compatibility with existing semiconductor technologies. That means they can be built using the same methods already used in the chip industry, making them easier to scale up than many competing quantum platforms.
“They can have long coherence times, up to 0.5 seconds, single-qubit gate fidelities exceeding 99.95%, and two-qubit gate fidelities surpassing the fault-tolerant threshold,” the authors write. That level of precision and stability is critical for real-world applications.
These qubits can also operate at temperatures above 1 Kelvin, making them some of the rare “hot qubits” that don’t require ultra-deep freezing. In some cases, fault-tolerant operations have even been achieved at this higher temperature, opening the door to more practical hardware designs.
Building Blocks and Types of Qubits
There are two main types of silicon spin qubits: gate-defined quantum dots and donor-based quantum dots.
In gate-defined systems, electrodes are placed on top of a semiconductor surface to shape an electric field that traps electrons from a two-dimensional electron gas. These electrons can then serve as qubits. The materials used include silicon, silicon/germanium blends, and metal-oxide-semiconductor structures.
Donor-based dots work differently. They use atoms like phosphorus as dopants, which means they place single impurity atoms into a silicon lattice. The electron associated with that atom is confined near the nucleus, and the spin of that electron—along with the spin of the nucleus—can be used to encode quantum information.
Both systems benefit from using isotopically purified silicon, which reduces unwanted nuclear interactions that could scramble the qubit. Their control and readout methods often rely on converting spin states into charge states, a process called spin-to-charge conversion. This makes it easier to detect and manipulate the qubits.
Electron spins in these systems have already demonstrated gate fidelities above the threshold needed for error correction. They can be operated using techniques like electron spin resonance or electric dipole spin resonance. Two-qubit gates are created through exchange interactions between nearby qubits, enabling logic operations like SWAP and controlled-NOT.
Connecting Qubits Across Distance
One of the key steps toward building a universal quantum computer is linking many qubits together over long distances. That way, the processor can scale up without requiring every qubit to be physically adjacent.
Scientists are making progress using techniques from circuit quantum electrodynamics, which use microwave photons to carry quantum information through superconducting resonators. Strong spin-photon coupling has been achieved using micromagnets that create synthetic spin-orbit interactions. This makes it possible to transfer quantum states between distant qubits, an essential feature for building multi-core quantum processors and distributed computing systems.
Hole spin qubits offer another option. These rely on the absence of electrons and have different physical characteristics, such as stronger spin-orbit coupling and weaker interaction with surrounding atoms. Some recent experiments have achieved single-qubit gate fidelities of 99.97% and two-qubit fidelities of 99.3%, along with strong coupling to photons. While this review focuses mainly on electron spins, hole spins are also being explored for their unique benefits.
Challenges and Future Directions
Even with all these advances, several challenges must be overcome. For gate-defined quantum dots, integrating the qubits with classical control electronics on the same chip remains a difficult task. Researchers also need to explore new layouts, possibly in two or three dimensions, to make better use of space and improve performance. Operating at higher temperatures would make the systems more practical, too.
For donor-based systems, new fabrication techniques must be developed to place atoms with atomic-scale precision. Integration with cryogenic control electronics and testing of new dopants could also improve performance and stability.
No matter the approach, scaling up quantum computers will require steady improvements in fidelity and reductions in noise. Researchers must address disorder in large qubit arrays and design better architectures that keep everything stable and easy to control.
Several research institutions and companies are already working to bring these ideas to life. Groups at Intel, CEA-Leti, HRL Laboratories, and IMEC are building foundry-compatible chips based on silicon qubits. These efforts, combined with advances in cryogenic CMOS technology, could one day lead to quantum systems on a single chip—a “quantum-classical hybrid” that merges quantum computing with traditional electronics.
Thanks to these efforts, what once seemed like a distant goal—the universal quantum computer—is slowly becoming a realistic target. Silicon spin qubits may well become the foundation of that future.
Note: The article above provided above by The Brighter Side of News.
Like these kind of feel good stories? Get The Brighter Side of News' newsletter.

Joshua Shavit
Science & Technology Writer | AI and Robotics Reporter
Joshua Shavit is a Los Angeles-based science and technology writer with a passion for exploring the breakthroughs shaping the future. As a contributor to The Brighter Side of News, he focuses on positive and transformative advancements in AI, technology, physics, engineering, robotics and space science. Joshua is currently working towards a Bachelor of Science in Business Administration at the University of California, Berkeley. He combines his academic background with a talent for storytelling, making complex scientific discoveries engaging and accessible. His work highlights the innovators behind the ideas, bringing readers closer to the people driving progress.